Investigation of PBI membranes for HT-PEMFCs
High-temperature polymer membrane fuel cells (HT-PEFCs)
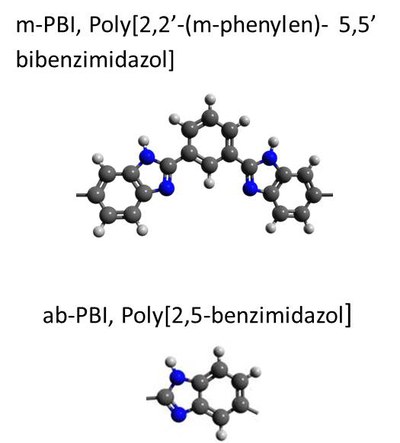
HT-PEFCs run at an operating temperature of about 160 °C with membrane electrode assemblies (MEAs), based on a polybenzimidazole-type polymer loaded with phosphoric acid (H3PO4) – e.g. m-PBI or ab-PBI (see Fig. 1). In the temperature range between 160 °C and 180 °C, the polymers loaded with H3PO4 display very high proton conductivity, relatively low gas permeability, and good mechanical stability.
The high operating temperature above 100 °C has several advantages over low-temperature polymer membrane fuel cells (LT-PEMFCs), which are limited to operating temperatures below 80 °C.
- The noble metal catalysts used in the cells (platinum) show a significantly higher tolerance to carbon monoxide poisoning. HT-PEFCs can be operated without the need for extensive fuel gas pretreatment with hydrogen, which is produced through reforming processes. This makes it possible to use PEMFCs in mobile and stationary applications where middle distillates such as kerosene or diesel are available as fuel reserves.
- During operation, the cells require no wetting of the gases. Cooling the cells (waste heat) is much easier and more effective due to the larger temperature difference.
- The waste heat from the cells can be used for other purposes in the overall system.
The system PBI – H2O –H3PO4
To further optimize performance and lifetime, detailed information about the ternary system PBI – H2O –H3PO4 in combination with conductivity measurements is required. The proton conductivity of a PBI membrane strongly depends on the H3PO4 and H2O content. At a given temperature, the H2O content is a function of the water vapor partial pressure. In HT-PEFCs, this pressure depends strongly on the current load and, due to limiting diffusion processes, on how it changes over time.
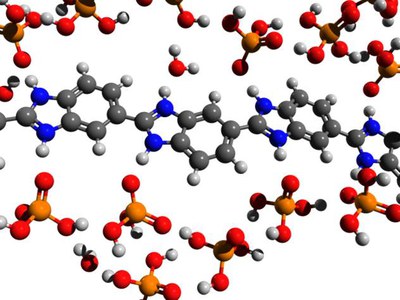
H3PO4 and other protic (acidic) electrolytes are bound to PBI-type polymer materials in a two-stage process (Fig. 2):
- protonation of the basic imidazole groups of the polymer chain by the electrolyte and formation of a “polymer salt” (1–2 electrolyte molecules per repeating unit). Bonding of the electrolyte anions via the electric charges.
- Uptake of more electrolyte molecules by means of hydrogen bridge bonds (up to 5 or 6 electrolyte molecules per repeating unit)
The formation of a cationic charged polymer chain 1) increases the osmotic pressure, which leads to the absorption of a large excess 2) of electrolyte molecules (and water molecules). This two-stage mechanism can be described with a BET-type absorption model (Guggenheim–Anderson–de Boer (GAB) isotherm). The equilibrium constants for the protonation i) and uptake via H bridges ii) differ by 3 orders of magnitude. [1-4]
Raman spectroscopic studies of electrolyte uptake
The individual stages of the adsorption isotherms can be correlated with Raman spectroscopic studies. Due to the protonation of the imidazole groups, strong changes of the spectrum occur in the range of the stretching vibrations of the aromatic rings and the N-H/C-H bending vibrations (1200–1650 cm-1). After taking up an equivalent amount of H3PO4 (stage i) for protonation, no further changes occur in this range. At very high loads, vibrational bands of free phosphoric acid can be observed [2, 3 , 5–7].
Understanding the interactions between electrolytes, such as phosphoric acid, and ionogenic polymers is the starting point for finding (link to PIL) alternative electrolytes for HT-PEFCs that can be absorbed in such polymer matrices.
References
[1] Majerus, A.; Conti, F.; Korte, C.; Lehnert, W.; Stolten, D. Thermogravimetric and Spectroscopic Investigation of the Interaction between Polybenzimidazole and Phosphoric Acid, ECS Trans. 2012, 50, 1155–1165.
[2] Korte, C.; Conti, F.; Wackerl, J.; Dams, P.; Majerus, A.; Lehnert, W. Uptake of Protic Electrolytes by Polybenzimidazole-Type Polymers – Absorption Isotherm and Electrolyte/Polymer Interactions, J. Appl. Electrochem. 2015, 45, 857–871.
[3] Korte, C.; Conti, F.; Wackerl, J.; Lehnert, W. 8 Phosphoric Acid and its Interactions with Polybenzimidazole-Type Polymers. In High Temperature Polymer Electrolyte Membrane Fuel Cells – Approaches, Status and Perspectives; Li, Q., Aili, D., Hjuler, H. A., Jensen, H. O., Eds.; Springer Verlag: 2016, 169–194.
[4] Conti, F.; Bertasi, F.; Wackerl, J.; Dams, P.; Noto, V. D.; Lehnert, W.; Korte, C. Phase Diagram Approach to Study Acid and Water Uptake of Polybenzimidazole-Type Membranes for Fuel Cells. ECS Trans. 2016, 72, 157–167.
[5] Conti, F.; Majerus, A.; Di Noto, V.; Korte, C.; Lehnert, W.; Stolten, D. Raman study of the polybenzimidazole-phosphoric acid interactions in membranes for fuel cells. Phys. Chem. Chem. Phys. 2012, 14, 10022–10026.
[6] Bettermann, H.; Labus, M.; Majerus, A.; Korte, C.; Lehnert, W. On-Line In-Situ Diagnostics of Processes Within HT-PEM Fuel Cells Membrane by Raman Microscopy. Proc. ASME. 2013, 55522, FuelCell2013-18155, pp. V001T01A008.
[7] Giffin, G. A.; Conti, F.; Lavina, S.; Majerus, A.; Pace, G.; Korte, C.; Lehnert, W.; Noto, V. D. A vibrational spectroscopic and modeling study of poly(2,5-benzimidazole) (ABPBI) – Phosphoric acid interactions in high temperature PEFC membranes. Int. J. Hydrogen Energy 2014, 39, 2776–2784.