Cells
Synthetic Cells: Controlling Shapes and Movements
Living cells are anything but rigid structures. They can take on many different forms, in order to move around, worm their way through narrow spaces, or to react to external stimuli. The outer sheath of cells – the lipid-bilayer membrane – has no active components. Instead, cell shapes and dynamic deformations are created in response to forces inside the cell. How are these forces generated, how does the membrane react to these forces, and which cell shapes are created and when?

These and similar questions, and the physical principles underlying these complex processes, can be explored with a new synthetic model system. Because the cell membrane does not play an active role in shaping cells, it can be replaced with simpler vesicles – tiny blisters whose flexible membrane is very similar to cell plasma membrane in structure and mechanical behaviour. Furthermore, the complex cytoskeleton is replaced by active, self-propelled particles.
Simulations of this model system allow the identification of three essential factors that determine the shape and dynamics of active vesicles: firstly, the membrane tension, secondly, the propulsive force of the active particles and thirdly, their concentration in the vesicle. The greatest variety of shapes are found to occurr at quite low particle concentrations. The results help to better understand biological processes and could be important for the development of synthetic cells, which might one day serve as miniature factories or work as micro-robots.
Reference:
Active particles induce large shape deformations in giant lipid vesicles; Nature 586, 52-56 (2020).
Artificial Cells and Soft Microrobots: Self-Organized Propulsion Controls Shape, Motility, and Sensing
Self-propulsion and navigation due to the sensing of environmental conditions—such as durotaxis and chemotaxis—are remarkable properties of biological cells that cannot be modeled by single- component self-propelled particles (like active Brownian particles).
Therefore, we introduce and study “flexocytes”, deformable vesicles with enclosed attached self-propelled pushing and pulling filaments that align due to steric and membrane-mediated interactions. These filaments mimic the action of actin filaments in cells, which show tread-milling motion or are displaced relative to each other by myosin motors.
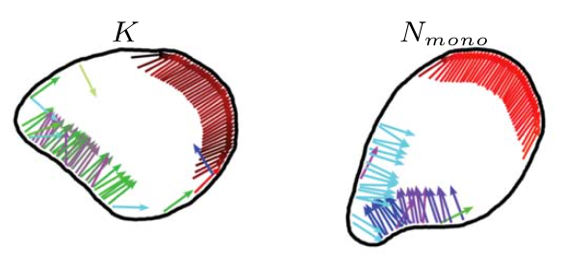
Using computer simulations in two dimensions, we show that the membrane deforms under the propulsion forces and forms shapes mimicking motile biological cells, such as keratocytes (K) and neutrophils (N). When interacting with walls or with interfaces between different substrates, the internal structure of a flexocyte reorganizes, resulting in a preferred angle of reflection or deflection, respectively. We predict a correlation between motility patterns, shapes, characteristics of the internal forces, and the response to micropatterned substrates and external stimuli.
We propose that engineered flexocytes with desired mechanosensitive capabilities enable the construction of soft-matter microrobots.
Reference:
C. Abaurrea-Velasco, T. Auth, and G. Gompper, Vesicles with internal active filaments: self-organized propulsion controls shape, motility, and dynamical response, New Journal of Physics 21, 123024 (2019)
How active are red blood cells?
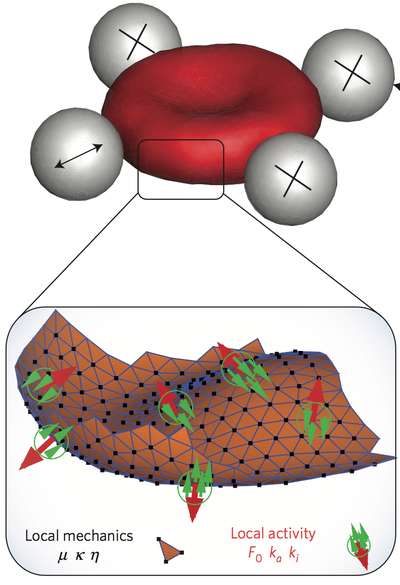
One of the first observations of red blood cells (RBC) by optical microscopy during the 19th century was the "vibrating" fluctuation of its plasma membrane . This phenomenon of flickering was first consider to be a signal of living matter, but later explained as thermal (passive) fluctuations of the cell membrane. Recent studies have indicated the involvement of non-equilibrium processes. However, the fundamental question whether membrane fluctuations are driven by an active process or simply by thermal agitation remains controversial.
Using active and passive microrheology to directly compare the membrane response and fluctuations on single erythrocytes, we have studied the violation of the fluctuation–dissipation relation, which is a direct demonstration of the non-equilibrium nature of flickering. Simulations show that several molecular mechanisms may explain the active fluctuations, and we predict their kinetics. Candidates are metabolic activity in the cytoskeletal spectrin network, and active force dipoles induced by ion pumps in the plasma membrane.
Minimal Model of Cell Motility
Actin polymerization is the mechanism that dominates cell motility in mesenchymal migration. Actin filaments attach via receptors to the substrate over which the cell is crawling, and polymerize/depolymerize at their plus/minus ends, which makes the filaments grow or shrink, and exert forces on the cell membrane. Due to the forces at the edge of a motile cell, the cell can move and change shape. For example, the aspect ratio of keratocytes increases with the ordering of the actin network and the actin density along the leading edge.

We propose a composite active particle -- consisting of quasi-two-dimensional self-propelled rods confined inside a rigid motile ring -- to study cell migration. Here, the basic idea is to mimic the dynamics and forces of cytoskeletal filaments by rod-like self-propelled particles: actin and microtubule treadmilling and polymerization forces are modeled by the self-propulsion of rigid rods with constant length. This system is studied by Brownian Dynamics simulations to elucidate the different kinds of motion that the complex self-propelled rings displays, which are persistent motion, random motion, circling, and run-and-circle motion -- depending on the rod-alignment interaction and the number of rods. Indeed, these motion patterns are also observed for motility of cells on a substrate.
For Microtubule Sliding, One Arm Is Better Than Two
The versatile cytoskeletal network in eukaryotic cells would be lifeless if not for kinesin motor proteins, which attach to and walk on microtubules. These motor proteins have arms that can crosslink adjacent polar filaments, and move along them. This causes the motors to stretch, and when multiple motors crosslink two microtubules, the cooperative effect of these motors results in a sliding stress that transports these microtubule relative to each other. In cells, this mechanism is important for cell-scale cytoplasmic flows, faster distribution of molecules and organelles, and the determination of cell shape.
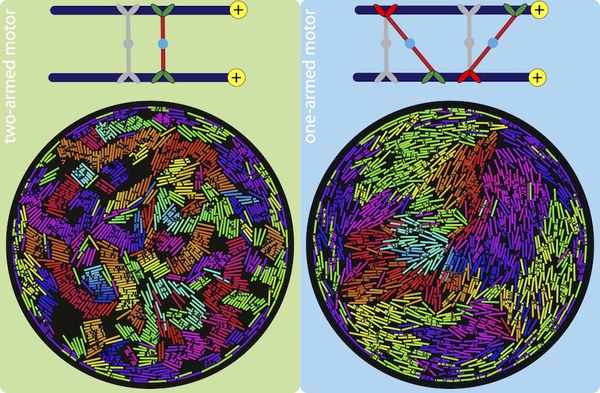
Using two-dimensional Brownian dynamics simulations, we study a dense, confined mixture of rigid microtubules (MTs) and motors that move unidirectionally on them. We show that one-arm motors, with a motile arm on only one of the two MTs, produce large polarity-sorted MT clusters, while two-arm motors, with motile arms on both microtubules, produce bundles. One-armed motors also induce, on average, higher velocities between anti-aligned MTs than two-armed motors. Our results, where MTs move faster near the confining wall, are consistent with experimental observations in Drosophila oocytes, where enhanced microtubule activity is found close to the confining plasma membrane.